C.-L. Shen1,2,3,4, H. Deshmukh1,*, J.M. Santos1,5,*, M.M. Elmassry6, P. Presto3,7, Z. Driver8, V. Bhakta8, V. Yakhnitsa7, T. Kiritoshi7, G. Ji3,7, J. Lovett1, A. Hamood9, V. Neugebauer2,3,7,10
1. Department of Pathology, Lubbock, TX 79430, USA; 2. Center of Excellence for Integrative Health, Lubbock, TX 79430, USA; 3. Center of Excellence for Translational Neuroscience and Therapeutics, Lubbock, TX 79430, USA, 79430, USA; 4. Obesity Research Institute, 8Department of Biochemistry, Texas Tech University, Lubbock, TX 79409, USA; 5. Woody L. Hunt School of Dental Medicine, Texas Tech University Health Sciences Center, El Paso, TX 79905, USA; 6. Department of Molecular Biology, Princeton University, Princeton, NJ 08540, USA; 7. Department of Pharmacology and Neuroscience, Lubbock, TX 79430, USA; 8. Department of Biochemistry, Texas Tech University, Lubbock, TX 79409, USA; 9. Department of Immunology and Molecular Microbiology, Lubbock, TX 79430, USA; 10. Garrison Institute on Aging, Texas Tech University Health Sciences Center, Lubbock, TX; * Equal contribution to the study.
Corresponding Author: Chwan-Li Shen, 1A096B, 3601 4th Street, Department of Pathology, Texas Tech University Health Sciences Center, Lubbock, Texas 79430-8115, USA. Telephone: +1 (806) 743-2815, Fax: +1 (806) 743-2117, E-mail: leslie.shen@ttuhsc.edu
J Frailty Aging 2024;13(4)319-330
Published online September 4, 2024, http://dx.doi.org/10.14283/jfa.2024.65
Abstract
Despite evidence linking the gut microbiome to neuropathic pain (NP), it is not known if altering gut microbiota can alleviate NP via the microbiome-gut-brain axis. This study examined if healthy gut microbiota of sham male rats (Sham+V) and dysbiotic gut microbiota of NP rats (spinal nerve ligation: NP, SNL+V) can be disrupted and restored, respectively, via fecal microbiota transplant (FMT) from the opposite group [Sham+(SNL-FMT) and SNL+(Sham-FMT), respectively]. All groups received FMT daily for two weeks, followed by three weeks without FMT. SNL rats showed higher mechanical hypersensitivity [SNL+V vs. Sham+V] throughout the study. After two weeks, the FMT of healthy gut microbiota decreased mechanical hypersensitivity in SNL rats [SNL+(Sham-FMT) vs. SNL+V]. A temporal shift in microbiome profiles after 2-week FMT treatment was observed in Sham+(SNL-FMT) and SNL+(Sham-FMT) groups, while the microbiome profile shifted back a certain extent after FMT ceased. At the end of study, the Sham+(SNL-FMT) group acquired low abundance of UCG-001, Odoribacter, and Peptococcaceae, and high abundance of UBA1819 and Victivallis. The SNL+(Sham-FMT) group maintained high abundance of Butyricimonas and Escherichia-Shigella. The SNL+(Sham-FMT) group had altered glial and macrophage activation/inflammation markers in the brain/colon than the SNL+V group. Relative to the SNL+V group, the SNL+(Sham-FMT) group had significantly lower gene expressions of GFAP (hypothalamus), IBA-1 (colon), and NF-κB (amygdala/colon), but higher gene expressions of complex I (amygdala/hypothalamus) and claudin-3 (amygdala/hypothalamus/colon). In conclusion, FMT containing healthy microbiota given to SNL rats attenuates mechanical hypersensitivity, modulates microbiota composition, and mitigates downstream glial activation/inflammation markers in a NP model. .
Key words: Neuropathic pain, gut microbiome, microbiota, neuroinflammation, brain, amygdala, rats, fecal transplant.
Introduction
Neuropathic pain (NP) management is a major clinical problem due to a lack of effective and safe treatment options (1). Studies suggest that gut microbiota may play a role in NP modulation via the gut-microbiota-brain axis. Gut microbiota represents the diversified microorganisms residing within the gastrointestinal tract (2). The brain can influence intestinal functions and gut microbial composition via the autonomic nervous system and the hypothalamic-pituitary-adrenal axis (3). The gut-microbiota-brain axis is a complex, multi-organ, bidirectional signaling system between the gut bacterial community and the brain; while not well understood, these interactions can involve neural pathways and transmitters, neuroimmune or neuroendocrine systems, and microbial metabolites (4-6).
In preclinical models of NP, gut microbiota depletion prevented or completely suppressed thermal hyperalgesia or mechanical allodynia in chronic constriction injury (CCI), oxaliplatin, and streptozocin-induced NP (7). Additionally, gut microbiota depletion prevented glial cell activation in the spinal cord of rats with CCI- or streptozocin-induced NP and suppressed inflammatory cytokine production in the dorsal root ganglion of rats with oxaliplatin-induced NP (7). The above studies demonstrate the distinct impact of gut microbiota in different forms of NP and may validate gut microbiota as a therapeutic or regulatory target for treating NP.
Fecal microbiota transplant (FMT) has received great attention as a tool to examine the influence of the gut’s microbiota in pain and pain-associated, depression-like phenotypes in rodents. For instance, FMT from spinal nerve injury rats with pain-induced depressive-like behaviors can affect such depression-like phenotypes in pseudo-germ-free mice (8). FMT from healthy rats has been shown to prevent both spinal cord injury-induced dysbiosis and the development of anxiety-like behaviors (9). However, no study has previously evaluated the role of FMT on pain sensitivity in rats in spinal nerve ligation (SNL)-induced NP. In our previous study, we demonstrated that the SNL procedure not only increased mechanical hypersensitivity but also caused dysbiosis in rats when compared to sham controls (10). However, it remains to be determined if (i) FMT from sham control rats can mitigate NP in SNL-injured rats, (ii) FMT from SNL-injured rats can induce NP in sham control rats, and (iii) the effect of FMT on NP persists after FMT ceases. The objective of this study was to determine if healthy gut microbiota of sham rats and dysbiosis of SNL rats can be disrupted and restored, respectively, via FMT from the opposite group. Therefore, the aims of the present study were (1) to evaluate the effects of FMT from sham control rats on mechanical hypersensitivity in SNL-operated rats, (2) to evaluate the effects of FMT from SNL rats on mechanical hypersensitivity in sham-operated rats, (3) to investigate the gut microbiota diversity and composition in rats due to FMT treatments, and (4) to evaluate whether FMT effects would continue after stopping FMT treatments for 3 weeks.
Furthermore, injury in the peripheral (PNS) or central nervous system (CNS) may lead to maladaptive changes in neurons, microglia cells (11), and astrocytes (12, 13). During NP progression, activation of microglial cells is imperative for the initiation and maintenance of pain and inflammation (14). Therefore, this study also explored the possible molecular mechanisms that may be associated with microglial/astrocyte activation and inflammation in the brain and colon of rats 3 weeks after FMT treatment. The focus is on the frontal cortex (right), amygdala (right central nucleus), and hypothalamus because these brain regions have been implicated in the emotional and cognitive aspects of pain; they have also been shown to exhibit bidirectional interactions between the gastrointestinal, neurological, and immune systems (6, 15, 16). Additionally, prior studies have elucidated evidence of right-hemispheric lateralization of amygdala function in pain, with neuroplasticity in the right amygdala serving pain-facilitating roles (17). Here, we hypothesized that FMT treatments would modulate pain behavior in NP rats by influencing the microbiome composition and suppressing glial activation and inflammation in the gut and brain.
Materials and Methods
Animals
All experimental procedures were approved by the Institutional Animal Care and Use Committee at Texas Tech University Health Sciences Center (IACUC protocol #21007). Male Sprague-Dawley rats (4-5 week-old, 150-180 g, Envigo, Cumberland, VA, USA) were individually housed under a 12-hour light-dark cycle with AIN-93G diet (catalog number: D10012G, Research Diets Inc., New Brunswick, NJ) and water ad libitum. Body weights, food intake, and water consumption were recorded weekly.
The first set of experiments consisted of two groups: (1) a control group that received a sham operation (n = 6) and (2) an NP group that received spinal nerve ligation (SNL) surgery (n = 6). Four weeks after SNL or sham surgery, the feces were collected from cecum and kept at -80°C for later fecal microbiota transplant (FMT). For the second set of experiments, two cohorts of rats were used and randomly divided into four experimental groups (n = 18): (1) sham with vehicle control gavage [Sham+V group, n = 4]; (2) sham with FMT from Experiment 1 SNL-operated rats [Sham+(SNL-FMT) group, n = 5]; (3) SNL with vehicle control gavage [SNL+V group, n = 4]; and (4) SNL with FMT from Experiment 1 sham-operated rats [SNL+(Sham-FMT) group, n = 5]. Rats in both Experiments 1 and 2 underwent identical experimental conditions, but only rats from Experiment 2 were used for the 16S rRNA amplicon analysis.
Stool pellets collected from Sham and SNL rats in Experiment 1 were pooled, respectively, and diluted to a concentration of 1:10 in PBS (10%, weight/volume), L-cysteine HCL (0.05%), glycerol (20%) and sterile water (60%), respectively (18, 19). The FMT or vehicle control treatments were given via oral gavage at a dose of 200 µL daily. All animals were gavaged with a fecal slurry (FMT: fecal microbiota transplant) or vehicle (PBS) at the time of the surgeries and for 2 weeks following surgeries. FMT or vehicle administration was discontinued in all groups for 3 weeks prior to sample collection.
Neuropathic pain induction
After 5 days of acclimatization, 9 animals were randomly assigned as sham-controls receiving sham surgery, while the remaining 9 animals underwent SNL surgery. The SNL model was used to induce NP in the left hind paw, as described in our previous work (10, 20). In brief, isoflurane was used for the induction (3%) and maintenance (2%) of anesthesia throughout the operation. After removing the left L6 transverse process, the left L5 spinal nerve was securely ligated with 6-0 silk thread. The paraspinal muscles were closed with sutures, and the skin was clipped together. Sham-operated animals served as controls for the NP model, receiving the same surgical steps without spinal nerve ligation. After surgery, all animals were given antibiotic treatment (1 dose of bacitracin, 8 mg/kg, subcutaneously, s.c.; VetOne, Boise, ID) and were monitored for any signs of infection or distress. Throughout the study period, the animals were monitored to reduce unnecessary stress or pain following ethical guidelines of the International Association for the Study of Pain (IASP) (21).
Assessment of pain behaviors in live animals
Mechanical withdrawal thresholds of spinal nocifensive reflexes were evaluated on the left hind paw using an electronic von Frey aesthesiometer (IITC Life Science, Woodland Hills, CA) with a plastic tip (catalog number 76-0488, Harvard Apparatus, Holliston, MA) in a dedicated testing area. SNL animals have been shown to develop mechanosensitivity within 7 days of surgery, which can persist for more than 10 weeks (22). Thus, we performed mechanosensitivity assessments at baseline (week 0, prior to the surgery), 1 and 2 weeks after receiving vehicle or FMT treatments following sham or SNL surgery, and 1, 2, and 3 weeks after stopping vehicle or FMT treatments following sham or SNL surgery. The average of six measurements per subject, taken at least 30 seconds apart, was calculated (10, 20).
Sample collection
Stool samples were collected for 16S rRNA amplicon sequencing at three timepoints (prior to FMT, 2 weeks after FMT, and 3 weeks after stopping FMT). At the end of the experiment, the animals were anesthetized with isoflurane (5%) and euthanized by decapitation. The brain regions [prefrontal cortex (right), amygdala (right central nucleus), and hypothalamus] and colon tissues were collected, immersed in liquid nitrogen, and stored at -80°C for later mRNA expression analysis.
RNA isolation and qRT-PCR
Total RNA was isolated from the amygdala (right central nucleus), frontal cortex (right), hypothalamus, and colon using the RNAzol RT (RN190, Molecular Research Center Inc., Cincinnati, Ohio, USA) and BAN (BN191, Molecular Research Center, Cincinnati, Ohio, USA) ratio at 1:200. Quantification of total RNA was performed by nanodrop (Nanodrop one, Thermo Scientific) at 260 nm wavelength and then reverse transcribed into cDNA using a thermal cycler (Bio-Rad S1000, Bio-Rad Laboratories, Inc., Hercules, CA, USA). qRT-PCR was performed on Quant Studio 12K Flex real-time PCR system (Life Technologies, 4470689, Carlsbad, CA, USA) for cDNA to amplify the target genes with Universal SYBR green supermix (Bio-rad Laboratories, Inc., 17251-24, Hercules, CA, USA). GFAP: forward primer 5’-AAT CTC ACA CAG GAC CTC GGC-3’ and reverse primer 5’-AGC CAA GGT GGC TTC ATC CG-3’. IBA-1: forward primer 5’-GAG CTA TGA GCC AGA GCA AGG ATT T-3’ and reverse primer 5’-ACT CCA TGT ACT TCG TCT TGA AGG -3’. NF-κB: forward primer 5’- CCT CCA CCC CGA CGT ATT GC-3’ and reverse primer 5’- GCC AAG GCC TGG TTT GAG AT-3’. Complex I: forward primer 5’-GGT TTG TCT ACA TCG GCT TCC – 3’ and reverse primer 5’-TAC AGA AGC TGG CGA TGC AAA -3’. Claudin-1: forward primer 5-GGC TGT CAT CGG GGG CAT AA -3’ and reverse primer 5’-CAG CAG CCC AGC CAG TAA AG -3’. Claudin-3: forward primer 5’-CCC AGC CTA CGG AGT TAC CC -3” and reverse primer 5”-TGC CGA TGA ATG CCG AAA CG -3’. β-actin: forward primer 5’-ACA ACC TTC TTG CAG CTC CTC C-3’ and reverse primer 5’-TGA CCC ATA CCC ACC ATC ACA-3’. The relative quantification of the gene expression was calculated with 2(ΔCT*1000) values normalized to respective β-actin value.
Gut microbiota profiling
DNA was isolated from feces using PowerFecal DNA isolation kit (Qiagen Inc., Germantown, MD, USA) following the manufacturer’s instructions. Amplicon sequencing of V4 variable region of the 16S rRNA gene was performed by MR DNA (Molecular Research LP, Shallowater, TX, USA). Briefly, the targeted variable region was amplified using PCR primers 515F/806R. Samples were multiplexed and pooled together in equal proportions based on their molecular weight and DNA concentrations. Pooled samples were purified using calibrated Ampure XP beads, then used in Illumina DNA library preparation. Sequencing was performed at MR DNA (www.mrdnalab.com, Shallowater, TX, USA) on a MiSeq following the manufacturer’s guidelines. Raw sequencing data have been deposited under BioProject accession numbers PRJNA000000 in the National Center for Biotechnology Information (NCBI) BioProject database.
Statistical analysis
The data is presented as the mean ± standard error of the mean (SEM) or mean ± standard deviation (SD). In order to address our research questions and a small sample size, data of von Frey tests and gene expression were analyzed by unpaired t-tests to compare between Sham+V group and Sham+(SNL-FMT) group or between SNL+V group and SNL+(Sham-FMT) group using GraphPad Prism 9 (GraphPad Software, San Diego, CA). Statistical significance was accepted at the level of p < 0.05. Additionally, we reported comparisons with a level of 0.05 < p < 0.1 to show a trend.
16S rRNA amplicon sequencing data was analyzed using QIIME 2 (23). In brief, reads were filtered, denoised, and merged. DADA2 was used to identify exact amplicon sequence variants (ASVs). For taxonomy assignment, Silva database release 138 database was used (24, 25). To compare the relative abundance of taxa between groups, we performed compositional analysis using LOCOM, a logistic regression model for testing differential abundance in compositional microbiome data with false discovery rate control (26). Statistical significance was accepted at the level of p < 0.05, unless stated otherwise. Visualization was performed in R version 4.0.5 (codename “Shake and Throw”).
Results
Mechanical hypersensitivity
At baseline (prior to surgeries), there was no difference in mechanical thresholds between all groups (Figure 1). Compared to the Sham+V group, administration of FMT from SNL to the Sham-operated rats [Sham+(SNL-FMT) group) did not affect mechanical hypersensitivity throughout the study period. On the other hand, relative to the SNL+V group, the SNL-operated rats that received sham feces [SNL+(Sham-FMT) group] showed significantly higher paw withdrawal thresholds as early as 1 week after initiating FMT treatment, suggesting antinociceptive effects of Sham-FMT treatment. Furthermore, this pain-mitigating effect in the SNL+(Sham-FMT) group was sustained for 3 weeks after stopping FMT treatment.
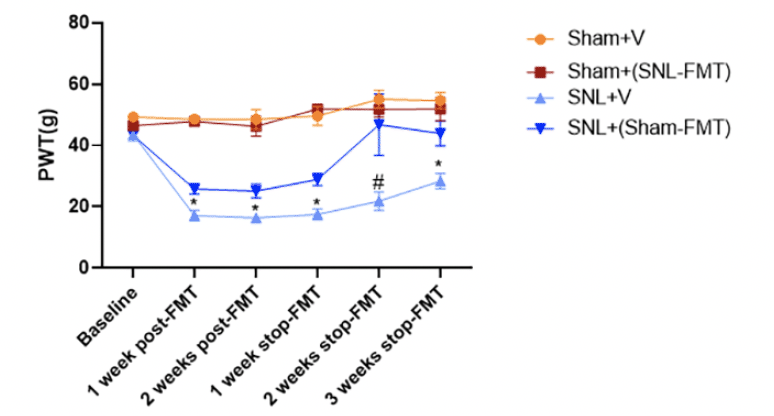
Figure 1. Effects of FMT treatment on mechanical hypersensitivity (paw withdrawal thresholds, PWT) in rats as assessed by an electronic von Frey (VFT) aesthesiometer
Data is expressed as mean ± SEM. n = 4 – 5 animals per group. Data obtained at the end of each week were compared between Sham+V and Sham+(SNL-FMT) and separately, between SNL+V and SNL+(Sham-FMT), using unpaired t-tests. * p < 0.05 SNL+V vs. SNL+(Sham-FMT). # 0.05 < p < 0.1 SNL+V vs. SNL+(Sham-FMT).
Gut microbiome diversity analyses
We first examined the beta-diversity among experimental groups using Weighted UniFrac distance measure (Figure 2A and 2B). Samples are labeled with 1 (baseline), 2 (2 weeks after FMT or vehicle treatments), or 3 (3 weeks after stopping FMT or vehicle treatments) according to their time point and colored based on their corresponding group. We observed a temporal shift in the microbiome profiles after 2-week FMT treatments (P ≤ 0.001, PERMANOVA). Overall, the strongest divergence in microbiome profiles from baseline was at the second time point, i.e., after 2 weeks of FMT treatments (Figure 2A). This is noticeable through the shift in the microbiome samples across PC1. This was only statistically significant in both FMT-treated groups [the Sham+(SNL-FMT) group and the SNL+(Sham-FMT) group] (P ≤ 0.05, pairwise PERMANOVA; Figure 2B), not in vehicle-treated groups (the Sham+V group and SNL+V group). 3 weeks after stopping FMT treatments, the microbiome profiles shifted back a certain extent. Again, this was only statistically significant in the case of the Sham+(SNL-FMT) group and the SNL+(Sham-FMT) group (P ≤ 0.05, pairwise PERMANOVA; Figure 2B). However, the microbiome in the Sham+(SNL-FMT) and SNL+(Sham-FMT) groups did not fully recover to their respective baseline profiles after stopping FMT treatments for 3 weeks (Figure 2B). Next, we examined alpha-diversity including richness and evenness (Figure 2C). While we did not observe a change in the evenness of microbiome species (P > 0.05, measured by Pielou’s evenness), we observed changes in the richness of microbiome species (p ≤ 0.05, measured by Faith’s phylogenetic diversity). After 2 weeks of post-FMT, except for the SNL+V group, richness dropped in the other 3 groups [Sham+V group, Sham+(SNL-FMT) group, and SNL+(Sham-FMT) group] (p ≤ 0.05). 3 weeks after stopping vehicle or FMT treatments (endpoint), the richness in different groups showed slight recovery, yet it never reached the baseline level (Figure 2C). This was exemplified in the Sham groups [the Sham+V group and the Sham+(SNL-FMT) group] and to a lesser extent in the SNL groups [the SNL+V group and the SNL+(Sham-FMT) group]. It is worth noting that while the richness in the SNL+V group at endpoint reached baseline level, that group’s baseline was significantly lower than other groups baseline (p ≤ 0.05). This baseline-diminished effect could be driven by the SNL treatment itself.
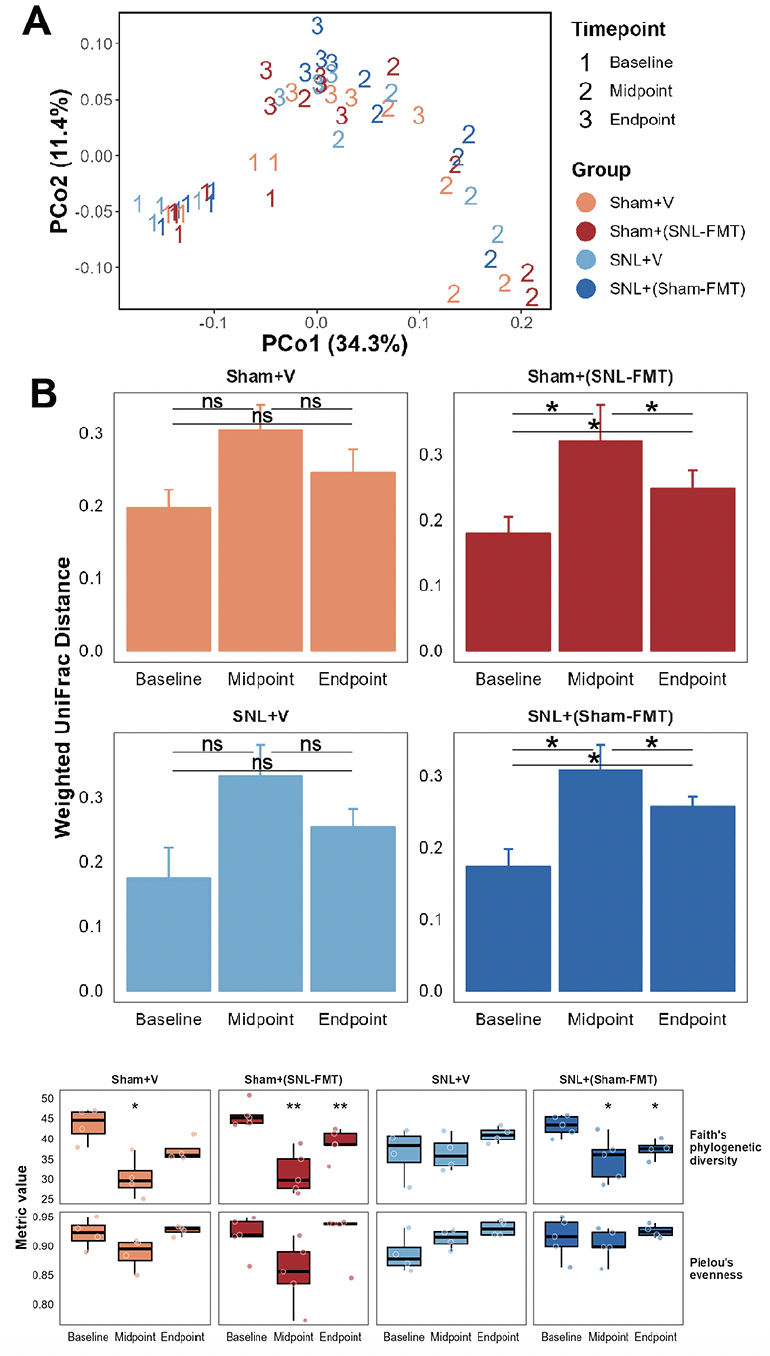
Figure 2. Effects of FMT treatments on the gut microbiome beta-diversity (A, B), alpha-diversity (C)
(A) Principal coordinate analysis (PCoA) using weighted UniFrac distance measure. (B) Weighted UniFrac distance measure examined temporally across all groups. Distance was calculated with respective baseline samples in each group. Mean and standard deviation are represented. Pairwise PERMANOVA, followed by false-discovery correction was used to determine statistical significance, * p ≤ 0.05. (C) Microbiome species richness was measured using Faith’s phylogenetic diversity and microbiome species evenness was measured using Pielou’s evenness. Mann-Whitney test was used to determine statistical significance, * p ≤ 0.05, ** p ≤ 0.01. Midpoint and Endpoint samples were compared to those at respective Baseline.
First, we investigated what species changed, even temporarily, in each experimental group (Figure 3A). This was to examine what changes occur in each group and how it relates to other groups. Overall, we reported more changes in groups receiving FMT treatments [Sham+(SNL-FMT) group and SNL+(Sham-FMT) group] than in those receiving vehicle treatments (Sham+V group and SNL+V group). Interestingly, there was a significant overlap across most groups. After stopping FMT treatments (endpoint), the abundance of microbiomes went back to their baseline in FMT-treated groups [Sham+(SNL-FMT) and SNL+(Sham-FMT)]. For instance, 3 weeks after stopping FMT treatment, the Sham+(SNL-FMT) group acquired (i) low abundance of UCG-001 (family Prevotellaceae), Odoribacter (family Marinifilaceae), and Peptococcaceae, and (ii) acquired high abundance of UBA1819 (family Ruminococcaceae) and Victivallis (family Victivallaceae). 3 weeks after stopping FMT treatment, the SNL+(Sham-FMT) group maintained high abundance of Butyricimonas (family Marinifilaceae) and Escherichia-Shigella (family Enterobacteriaceae).
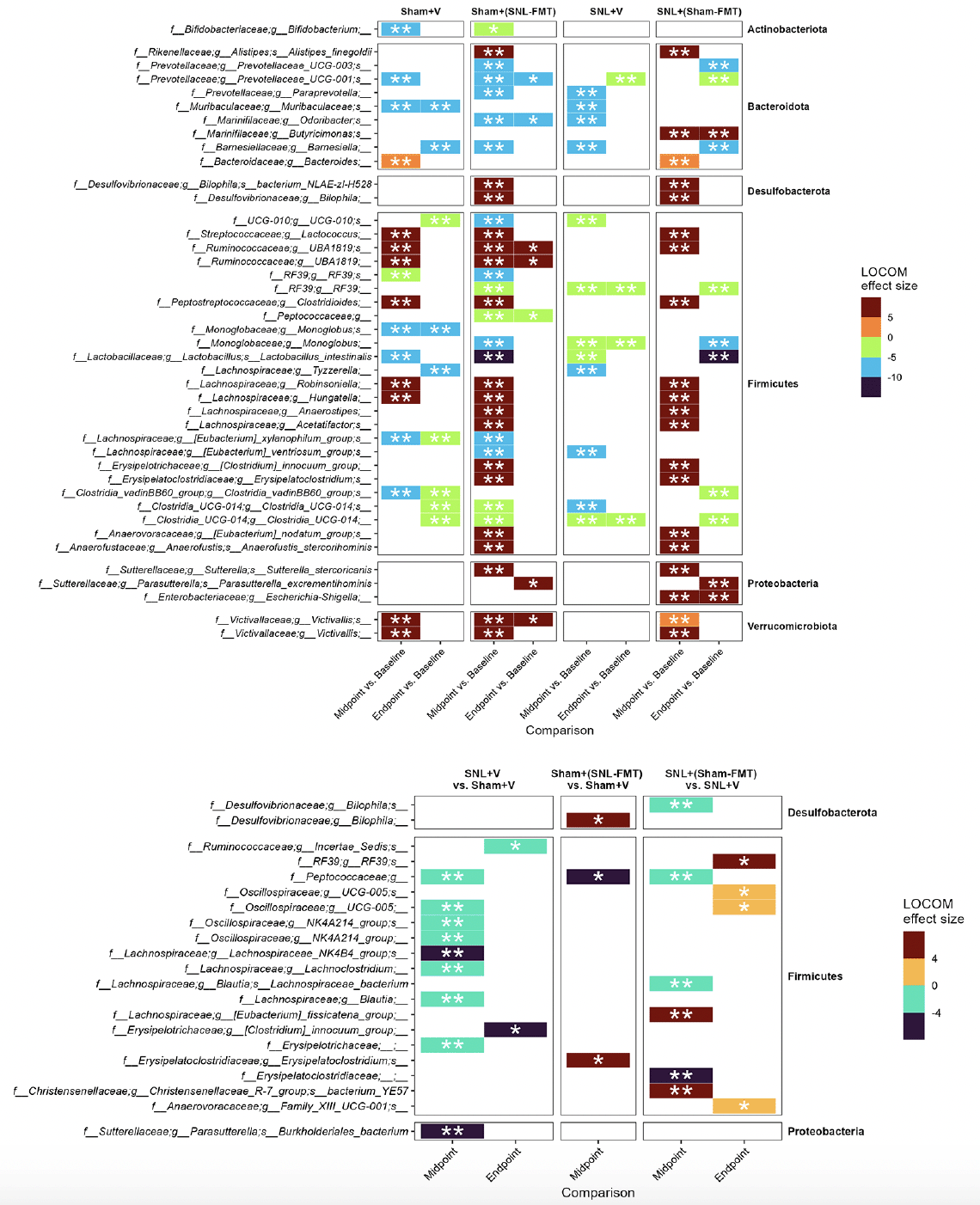
Figure 3. Microbiome composition changes between timepoints, i.e., midpoint vs. baseline and endpoint vs. baseline, in each group (A) and changes between groups (B)
LOCOM analysis was used to determine statistical significance followed by Benjamini-Hochberg Procedure. * padj ≤ 0.05, ** padj ≤ 0.01. Missing timepoint columns indicates that no statistical significance was observed in the corresponding comparison, e.g., Sham+(SNL-FMT) group vs. Sham+V group at Endpoint.
To decipher the link between FMT treatment effects in SNL-operated animals on microbiomes and on mechanical hypersensitivity, we took several approaches. All of them were based on LOCOM analysis to identify microbiome species of differential relative abundance across animal groups and time points. We determined what species change at each time point between the SNL+V group and the Sham+V group as well as between the SNL+(Sham-FMT) group and the SNL+V group (Figure 3B). This was based on the hypothesis that the SNL surgery changes the microbiome species in a way that influences mechanical hypersensitivity, and that such effects might be reversed with FMT treatment. Among all microbiome species, only UCG-005 (family Oscillospiraceae) exhibited such a reversal through its decrease in the SNL+V group and increase with FMT treatment that was received from sham-operated animals at midpoint. The abundance of UCG-005 was negatively associated with the degree of mechanical hypersensitivity. Then, we determined what species change at each timepoint between Sham+(SNL-FMT) group vs. Sham+V group as well as between SNL+(Sham-FMT) group vs. SNL+V group (Figure 3B). This is based on the hypothesis that the changes we observed with SNL+(Sham-FMT) rats would not overlap or exist with Sham+(SNL-FMT) rats. Among the top genus, (i) UCG-005 (family Oscillospiraceae) increased with SNL+(Sham-FMT) group, but not with Sham+(SNL-FMT) group at midpoint; and (ii) Bilophila (family Desulfovibrionaceae) was increased in the Sham+(SNL-FMT) group, but it was decreased in the SNL+(Sham-FMT) group at midpoint. There was no statistical significance in the corresponding comparison, e.g., Sham+(SNL-FMT) group vs. Sham+V group at baseline or endpoint (data not shown). The abundance of UCG-005 and Bilophila was negatively and positively associated with the degree of mechanical hypersensitivity. Finally, we examined the species that surfaced from our analyses and their trends in different groups over time and if they mirrored what we observed with the mechanical hypersensitivity trend (Figure 4). Specifically, based on the mechanical hypersensitivity data, we expected to see changes in the microbiome of the SNL+V group that were quite different than the other 3 groups [Sham+V group, Sham+(SNL-FMT) group, and SNL+(Sham-FMT) group]. For example, UCG-005 showed a promising trend as it was quite different in the SNL+V group than the other 3 groups with an increase over time. In contrast, Victivallis increased in the SNL+V group while it stayed low in other 3 groups. To further corroborate our results, we performed a volatility analysis to investigate the microbiome changes longitudinally using QIIME2 (27). We examined the changes in microbiome ASVs and filtered those with importance metric ≥ 0.001 to focus on the most significant changes over time. This resulted in identifying 32 ASVs of high importance (Figure 5). While the analysis indicated the change in ASVs across all groups, several ASVs changed in a trend mirroring our previous analysis, e.g., the decrease in Odoribacter (family Marinifilaceae) and Victivallis (family Victivallaceae) in SNL+(Sham-FMT).
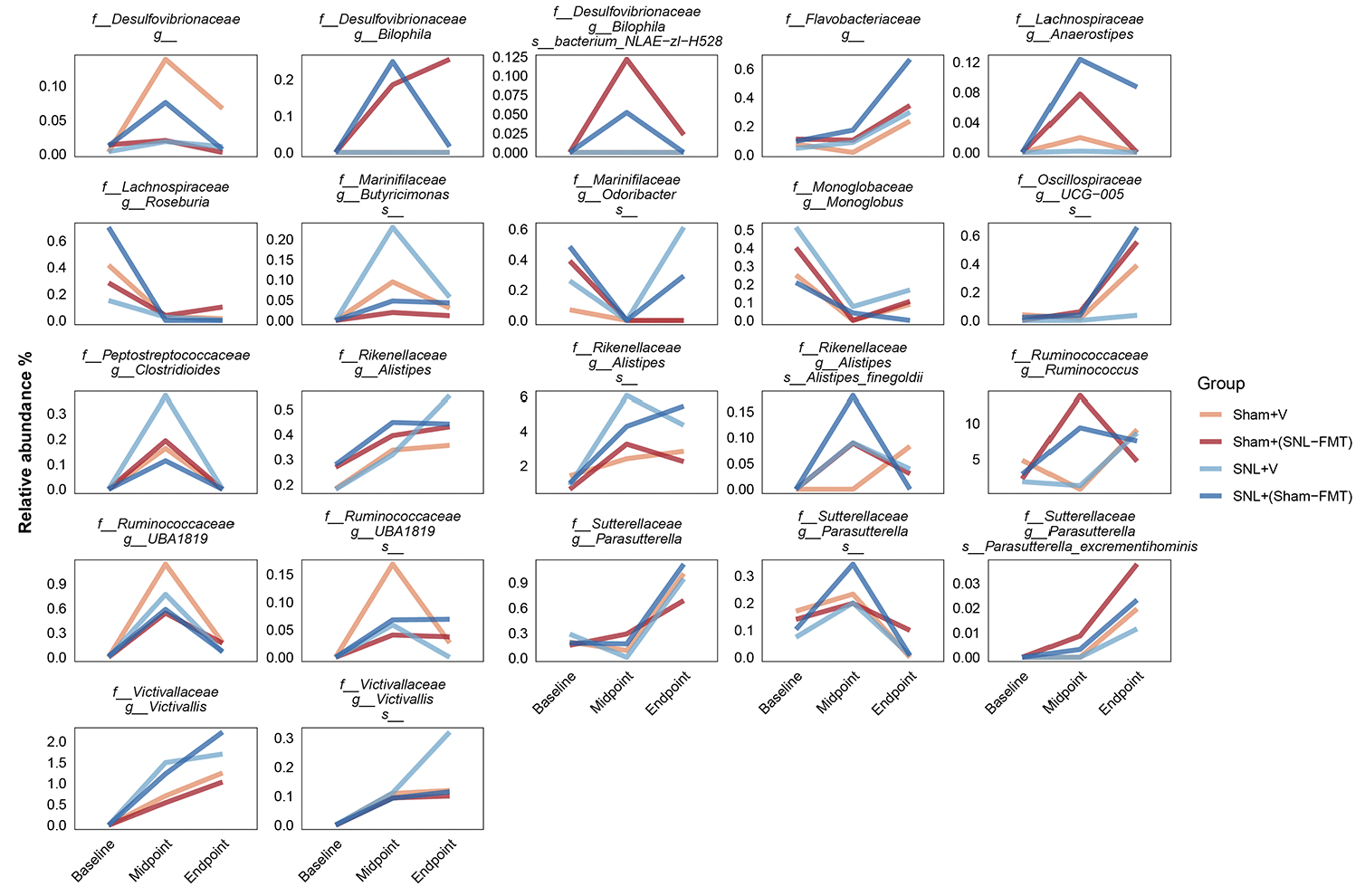
Figure 4. Selected microbiome species and their relative abundance temporally across groups. Mean is represented for each group
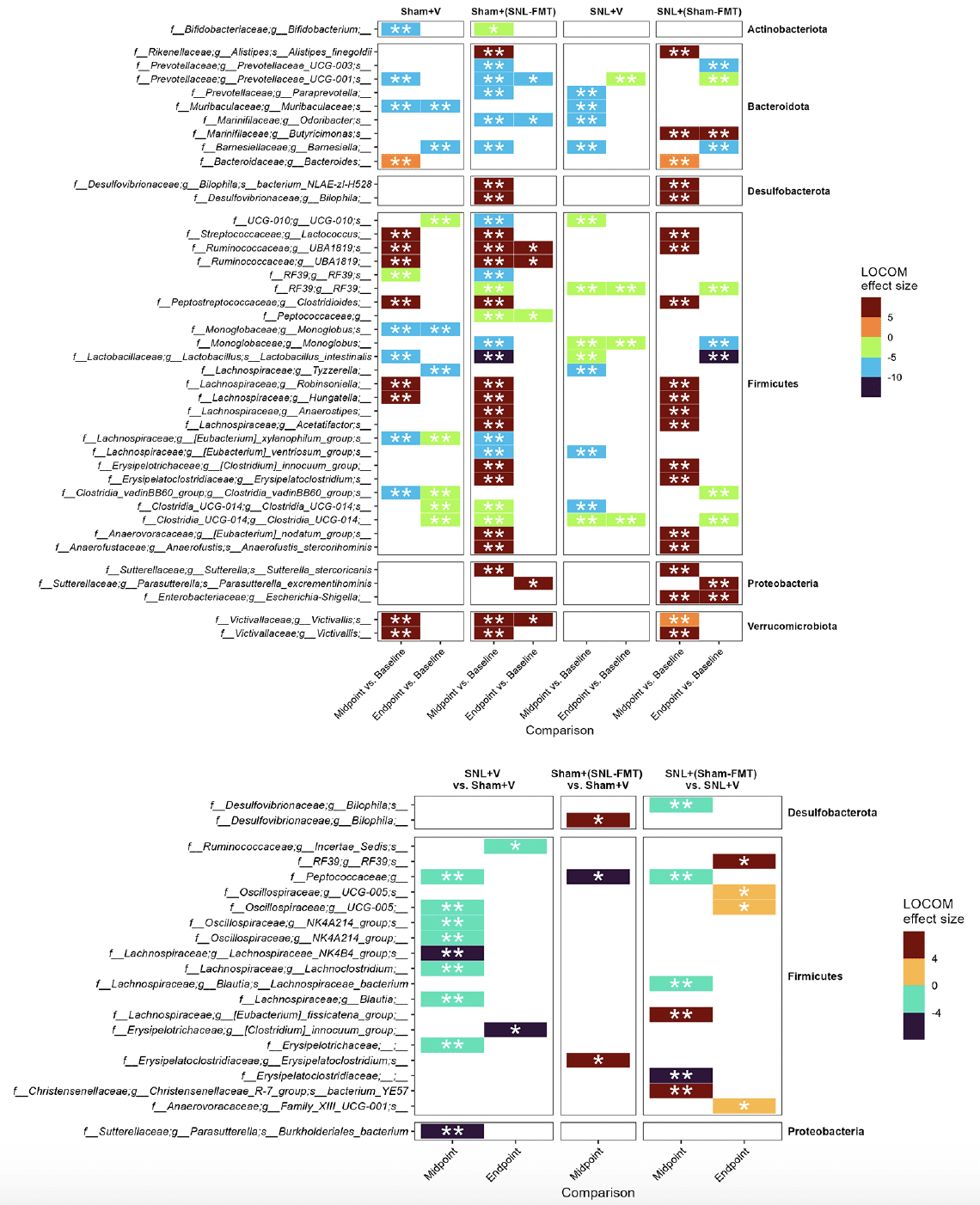
Figure 5. Temporal changes in the microbiome species identified from volatility analysis (importance ≥ 0.001)
Mean is represented for each group.
Modulation of microglial/astrocyte activation and inflammation in brain and colon
We evaluated the effects of FMT treatments on GFAP mRNA expression, a marker of CNS astrocytes and enteric glia cells, in brain regions relevant to pain modulation and gut-brain interactions (see Introduction) in the colon (Figure 6A). Relative to the Sham+V group, the Sham+(SNL-FMT) group had higher GFAP mRNA expression levels in all 3 brain regions (amygdala, frontal cortex, and hypothalamus) of rats. Compared to the SNL+V group, the SNL+(Sham-FMT) group had lower GFAP mRNA expression levels in the hypothalamus of rats. FMT treatments did not affect the GFAP mRNA expression in the colon. Next, we evaluated the effects of FMT treatments on mRNA expression of IBA-1, a biomarker of microglial activation (Figure 6B). Compared to the Sham+V group, the Sham+(SNL-FMT) group had higher IBA-1 mRNA expression levels in the frontal cortex and colon of rats. Conversely, the SNL+(Sham-FMT) rats had lower IBA-1 mRNA expression levels in the colon than the SNL+V group. We also examined the effects of FMT treatments on the inflammatory marker NF-κB, an mRNA expression in the brain and colon tissues (Figure 6C). Relative to the Sham+V group, the Sham+(SNL-FMT) group had higher NF-κB mRNA expression levels in the amygdala and colon of rats. Conversely, when compared to the SNL+V rats, the SNL+(Sham-FMT) rats had lower NF-κB mRNA expression levels in the amygdala and colon.
Effects of FMT treatments were measured on complex I mRNA expression for mitochondrial respiratory chain complexes (Figure 6D). After FMT treatment, the complex I mRNA expression was significantly decreased in the amygdala, hypothalamus, and colon of the Sham+(SNL-FMT) rats (p < 0.05). Conversely, the complex I mRNA expression increased in the amygdala (p < 0.05) and hypothalamus (0.05 < p < 0.1) of SNL+(Sham-FMT) rats compared to those in the SNL+V group. Finally, we evaluated the effect of FMT treatments on the mRNA expression of the tight junction protein markers claudin-1 (Figure 6E) and claudin-3 (Figure 6F) in the brain and colon tissues. Compared to the Sham+V group, the Sham+(SNL-FMT) group had lower claudin-1 mRNA expression levels in the amygdala and hypothalamus. Conversely, SNL+(Sham-FMT) rats had increased claudin-1 mRNA expression levels in the amygdala and frontal cortex compared to the SNL group. Similar to the claudin-1 mRNA expression, the Sham+(SNL-FMT) group had lower claudin-3 mRNA expression in the amygdala compared to the SNL+V group, whereas the SNL+(Sham-FMT) group had increased claudin-3 mRNA expression in the amygdala, hypothalamus, and colon of rats.
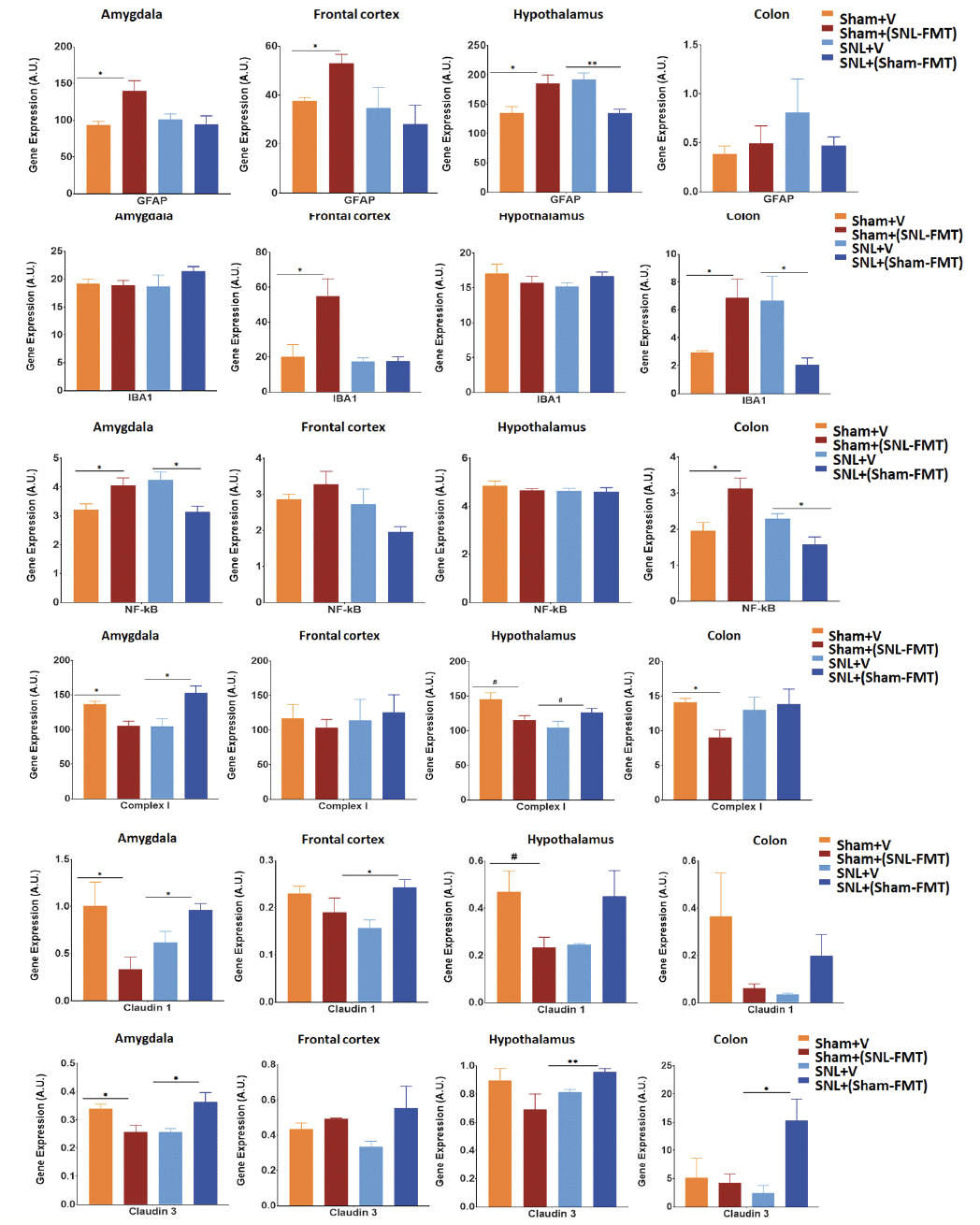
Figure 6. Effects of FMT treatment on mRNA expression of GFAP (A), IBA-1 (B), NF-kB (C), Complex I (D), Claudin-1 (E) and Claudin-3 (F) in the amygdala, frontal cortex, hypothalamus, and colon of rats
Data is expressed as mean ± SEM. Data was analyzed by unpaired t-test; Sham+V vs. Sham+(SNL-FMT) and SNL+V vs. SNL+(Sham-FMT). * p < 0.05, ** p < 0.005.
Discussion
To the best of our knowledge, this is the first study demonstrating that FMT treatments showed differential effects on mechanical hypersensitivity and the role of gut microbiota in neuropathic pain (SNL rat model). Even after stopping FMT, the FMT-induced beneficial gut microbiota changes appeared to persist.
The microbiota in the gut regulate central sensitization (28). Altered gut microbiota profiles of rats (vehicle or FMT treatments) provided an opportunity to analyze the association between pain-related behaviors and inflammatory responses in NP rats. In the present study, the abundance analysis results showed that UGC-005 and Bilophila might exert measurable effects on the development of SNL-induced NP. In the present study, at the genus level, the finding that Oscillospiraceae UCG-005 was significantly increased in the SNL+(Sham-FMT) group compared with the SNL+V group differs from a previous study (29) using paclitaxel-induced peripheral neuropathy (PTX) before FMT treatment. That study reported that (i) the abundance of UCG-005 was elevated in the animals with PTX compared to those in the vehicle group; and (ii) relative to the PTX plus antibiotics group or PTX plus FMT from vehicle animals, the PTX group had greater abundance of UCG-005 along with greater mechanical hypersensitivity (29). The discrepancy in UCG-005 between the studies could be due to different NP models (SNL in ours vs. PTX in Shi’s) and usage of antibiotics (no antibiotics in ours vs. antibiotics use in Shi’s). Interestingly, the abundance of Bilophila in our study showed the opposite trend in the Sham+(SNL-FMT) group (increased Bilophila) with greater mechanical hypersensitivity and in the SNL+(Sham-FMT) group (decreased Bilophila) with less mechanical hypersensitivity due to FMT treatments. The positive correlation of abundance of Bilophila with mechanical hypersensitivity is consistent with a cohort study showing that a greater relative abundance of the genera Bilophila was positively associated with pain, such as symptomatic hand osteoarthritis pain (30).
Neuropathic pain that originates from lesions or diseases of the nervous system can lead to extensive neuroplastic changes in the brain and is often associated with extensive neuroinflammation and glial cell activation. Microglia and astrocytes are key components of the central nervous system and innate immune system that have been shown to both influence and respond to modified neuronal activity within nociceptive pathways (31, 32). Their complex interactions with neurons contribute to synaptic modulation and neuroplasticity. Key proinflammatory mediators released by activated microglia include cytokines such as interleukin-1β (IL-1β), interleukin-6 (IL-6), and tumor necrosis factor-α (TNFα). Other substances implicated in neuron-to-glia signaling include mitochondrial complex I, nitric oxide, and brain-derived neurotrophic factor (BDNF). In response, nearby astrocytes have been shown to become reactive and release pro- and anti-inflammatory cytokines, perpetuating neuroinflammation-related signaling. Current knowledge regarding neuroimmune signaling in pain conditions largely stems from research on peripheral and spinal nociceptive processing, whereas little is known about similar processes in brain regions associated with different aspects of pain (32-34). The gut microbiota has been linked to both pro- and anti-inflammatory microglial activation states, and microglia dysfunction has been shown to trigger signaling cascades of neuroinflammation (35-37 ).
Transplanting the gut microbiota from nerve-injured ‘donor’ mice into naïve recipients can transmit pain hypersensitivity, indicating the gut microbiota help drive neuropathic pain pathology. It has previously been found that FMT attenuated peripheral neuropathy (obesity-induced mechanical allodynia and thermal hyperalgesia) and induced changes in the immune cell populations of the PNS through the modulation of butyrate (27), a circulating short-chain fatty acid, that has previously been linked to the improvement of pain-associated behaviors in NP models (38). With particular regard to FMT, it has been demonstrated that circulating butyrate secreted by the gut microbiome underlies the effect of FMT (27). Previous studies suggest that butyrate regulated inflammatory cytokine expression in microglia and astrocytes (39-41). In addition, Wei et al. reported that butyrate suppressed microglia-mediated neuroinflammation in the brain through the CPR109A/PPAR-γ/TLR4-NF-κB signaling pathways (41). Though we did not explore short-chain fatty acids in this study, the published studies suggest that the presence of short-chain fatty acids, such as butyrate, would play an important role in brain and their subsequent influence in the neuroimmune process of NP. The present findings of this study highlight the potential impact of FMT on neuroimmune-related signaling mechanisms within the brain, which may be distinct from NP-related processes, and emphasize the need for glial subtype-specific investigation in both conditions to further elucidate functional consequences of glial activation.
In the current study, we report (i) upregulated GFAP mRNA expression levels in the amygdala, frontal cortex, and hypothalamus of Sham+(SNL-FMT) rats and (ii) downregulated GFAP mRNA expression levels in the hypothalamus of SNL+(Sham-FMT) rats. FMT treatment received from the Sham-treated rats suppressed SNL-induced microglial activation and inflammation in the brain and colon of SNL rats. This study is the first to show that FMT treatments had differential effects on glial activation and inflammation in different brain regions (amygdala, frontal cortex, and hypothalamus) through a brain-gut interaction. Through microbial metabolites, gut microbiota interacts with dorsal root ganglia and the spinal cord (42, 43). Several mediators (i.e., IL-1β, TNF-α, short-chain fatty acids, and bile acids) have been linked to the development of NP by regulating peripheral and central sensitization (14, 44). Activation of astrocytes and microglial cells promotes the release of cytokines and chemokines, altering glutamatergic and GABAergic neurotransmission and eventually leading to pain hypersensitivity. While neuroimmune signaling in the spinal cord is well-established as a pain mechanism (32), little is known about pain-related neuroimmune signaling in the brain. Recent work from our team has found increased astrocyte activation in the amygdala in SNL-induced NP, which was associated with decreased pain-related behaviors and improved pain-associated neuronal functions, suggesting a protective role for astrocytes in this region (45, 46). It is important to note that further astrocyte subtypes (i.e., pro- versus anti-inflammatory cells) that may contribute to distinct astrocytic functional states in the amygdala were not explored (45). The findings of this study illustrate that markers of global astrocyte activation do not fully encompass pain-related behavioral differences, suggesting that the use of additional markers is warranted to differentiate between astrocyte subtypes and to further investigate their specific roles within the brain in NP. On the other hand, inhibition of microglia in the amygdala has previously been linked to a decrease in pain-related behaviors in an irritable bowel syndrome model (47) and to delayed pain-related behaviors in a NP model (48). However, the present findings suggest that amygdala microglia activation may not fully explain NP-related behaviors. Future studies are warranted to explore distinct microglial subtypes and its associated functional states.
A “leaky gut” refers to a damaged gut lining that loses function as a barrier, leading to an increase in the permeability of the intestinal mucosa and effecting low-grade inflammation (8, 49). The connection between a leaky gut and neuroinflammation in NP and NP-associated behaviors has received increased consideration, and accumulating evidence suggests that changes in the permeability of the intestines and the blood-brain barrier enhance the entrance of microbiota-produced substances into the PNS and CNS (8, 49, 50). Downregulation of claudin within the brain has previously been associated with abnormal neuronal activity and linked to permeability changes within the blood-brain barrier (51). A prior study by our group (52) demonstrated that relative to Sham-treated rats, NP rats 4 weeks after SNL induction had increased intestinal permeability (leaky gut), as measured by the increased ratio of lactulose/D-mannitol concentration in the urine. At the molecular level, compared to control rats, rats with diabetic neuropathy had significant lower gene expression of claudin-3 (tight junction protein) in the colon (53). In the present study, the findings that the SNL+(Sham-FMT) group) had (i) reduced mechanical hypersensitivity and (ii) increased claudin-3 mRNA expression in the amygdala, hypothalamus, and colon (compared to those in the SNL-V group) suggest that FMT treatment mitigated pain when the leaky gut of NP rats was repaired. Furthermore, although FMT treatment had no effect on mechanical hypersensitivity in the Sham+(SNL-FMT) group, these animals had decreased claudin-1 or claudin-3 mRNA expression in the amygdala, indicating a detrimental impact of FMT collected from SNL; the data suggests that intestinal changes induced in normal animals with intact intestinal are not sufficient to generate NP behaviors. This study examined how FMT treatments affect the changes in mRNA expression levels of claudin-1 and claudin-3 (marker of tight junction protein) in the brain and colon. In order to confirm links between a leaky gut (intestinal permeability), blood-brain barrier integrity, and tight junction protein expression, future studies are warranted to assess not only mRNA expression but also protein expression of tight junction proteins in the brain and colon, and to link the gut and brain more directly using more detail gain- and loss-of-function approaches.
There are limitations in the present study as follows. The small sample size could affect significances due to the variation within the same group. Future studies with a larger sample size and additional pro- and anti-inflammatory markers to assess distinct microglia and astrocyte functions are warranted to confirm the current findings. While the study’s focus on NP is clear, the inclusion of emotional or cognitive behavioral alterations could provide a more comprehensive understanding of the gut-brain axis’s role. Future studies should include both emotional and cognitive behaviors in a larger sample size of study to corroborate that if behavioral abnormalities alongside SNL and changes with Sham-FMT or sham+(SNL-FMT) can be observed. Such findings would significantly contribute to the discourse on gut microbiota’s role in neurophysiology and behavioral modulation. Only male animals used in this study, considering the complex nature of biological system and the presence of sex differences in the modulation of pain (54-56), it is plausible to hypothesize that there may be sex differences in the FMT-mediated analgesic effects along with molecular mechanisms. Future studies should employ the same FMT approaches in female NP rats to corroborate the sex difference in the FMT-medicated analgesic effects and altered transcripts of brain and colon. In this study, we did not use antibiotics in animals prior to FMT treatments for several reasons. Antibiotics are used to deplete the inhabiting microbiota to enable the engraftment of the introduced microbiota. However, one big caveat is that antibiotics themselves influence host behavior, including food intake, underlying physiology, pain sensitivity, and pain-associated behavior (49, 57), Furthermore, literature suggests that antibiotic abstinence still allows for microbiota engraftment (58). Indeed, we observed changes in the microbiota of rats after FMT, indicating successful engraftment. This also led to decreased mechanical hypersensitivity in SNL rats treated with Sham-FMT.
In conclusion, our results show beneficial effects of FMT from sham control animals on pain behaviors, gut microbiota, and several markers of inflammation in the brain and colon of neuropathic rats (SNL model). FMT from neuropathic rats was not sufficient to induce neuropathic pain-like behaviors in sham control rats, although several parameters of gut microbiota and inflammation in the colon and brain were changed. Additional outcome measures of NP behaviors are needed to confirm or resolve this connection. The present study provides the scientific premise for mechanistic studies into the causality and mechanisms of gut microbiome-brain interactions in NP.
Author Contributions: CLS, HD, JMS, MME, PP, VN contributed to the conceptualization of the study design and manuscript preparation. CLS, MME, and VN contributed manuscript draft. PP contributed to pain induction surgery. VB and ZD contributed to daily oral gavage of treatments and animal maintenance. JMS contributed to pain-associated behaviors assessment and analysis, DNA extraction from cecum feces, and preparation of FMT solutions for the treatments after surgery. ZD contributed to mRNA extractions and cDNA conversions. HD contributed to qRT-PCR and gene expression analysis. MME contributed to gut microbiome data analysis and interpretation. JMS, VY, TK, GI, and JL contributed sample collection and data interpretation. All authors have read and agreed to the published version of the manuscript. The authors declare that there is no conflict of interest.
Acknowledgment: This work is supported by USDA-NIFA 2021-67017-34026 (CLS/VN). The work in the VN’s laboratory was supported by NIH grant R01NS038261. Real-time PCR data was generated in the Molecular Biology Core Facility supported in part by TTUHSC.
Institutional Review Board Statement: Not applicable.
Informed Consent Statement: Not applicable.
Data Availability Statement: Data will be shared upon request.
Conflicts of Interest: The authors declare no conflict of interest.
Open Access: This article is distributed under the terms of the Creative Commons Attribution 4.0 International License (http://creativecommons.org/licenses/by/4.0/), which permits use, duplication, adaptation, distribution and reproduction in any medium or format, as long as you give appropriate credit to the original author(s) and the source, provide a link to the Creative Commons license and indicate if changes were made.
References
1. Finnerup NB, Attal N, Haroutounian S, McNicol E, Baron R, Dworkin RH, et al. Pharmacotherapy for neuropathic pain in adults: a systematic review and meta-analysis. Lancet Neurol. 2015;14(2):162-173.
2. Dinan TG, Cryan JF. Microbes, Immunity, and Behavior: Psychoneuroimmunology Meets the Microbiome. Neuropsychopharmacology. 2017;42(1):178-192.
3. Rhee SH, Pothoulakis C, Mayer EA. Principles and clinical implications of the brain-gut-enteric microbiota axis. Nat Rev Gastroenterol Hepatol. 2009;6(5):306-314.
4. Fung TC, Olson CA, Hsiao EY. Interactions between the microbiota, immune and nervous systems in health and disease. Nat Neurosci. 2017;20(2):145-155.
5. Cussotto S, Sandhu KV, Dinan TG, Cryan JF. The Neuroendocrinology of the Microbiota-Gut-Brain Axis: A Behavioural Perspective. Front Neuroendocrinol. 2018;51:80-101.
6. Li X, Chen LM, Kumar G, Zhang SJ, Zhong QH, Zhang HY, et al. Therapeutic Interventions of Gut-Brain Axis as Novel Strategies for Treatment of Alcohol Use Disorder Associated Cognitive and Mood Dysfunction. Front Neurosci. 2022;16:820106.
7. Ma P, Mo R, Liao H, Qiu C, Wu G, Yang C, et al. Gut microbiota depletion by antibiotics ameliorates somatic neuropathic pain induced by nerve injury, chemotherapy, and diabetes in mice. J Neuroinflammation. 2022;19(1):169.
8. Yang C, Fang X, Zhan G, Huang N, Li S, Bi J, et al. Key role of gut microbiota in anhedonia-like phenotype in rodents with neuropathic pain. Transl Psychiatry. 2019;9(1):57.
9. Schmidt EKA, Torres-Espin A, Raposo PJF, Madsen KL, Kigerl KA, Popovich PG, et al. Fecal transplant prevents gut dysbiosis and anxiety-like behaviour after spinal cord injury in rats. PLoS One. 2020;15(1):e0226128.
10. Shen CL, Wang R, Ji G, Elmassry MM, Zabet-Moghaddam M, Vellers H, et al. Dietary supplementation of gingerols- and shogaols-enriched ginger root extract attenuate pain-associated behaviors while modulating gut microbiota and metabolites in rats with spinal nerve ligation. J Nutr Biochem. 2022;100:108904.
11. Karavis MY, Siafaka I, Vadalouca A, Georgoudis G. Role of Microglia in Neuropathic Pain. Cureus. 2023;15(8):e43555.
12. Ji RR, Donnelly CR, Nedergaard M. Astrocytes in chronic pain and itch. Nat Rev Neurosci. 2019;20(11):667-685.
13. Dos Santos R, Veras F, Netto G, Elisei L, Sorgi C, Faccioli L, et al. Cannabidiol prevents chemotherapy-induced neuropathic pain by modulating spinal TLR4 via endocannabinoid system activation. J Pharm Pharmacol. 2023;75(5):655-665.
14. Chen G, Zhang YQ, Qadri YJ, Serhan CN, Ji RR. Microglia in Pain: Detrimental and Protective Roles in Pathogenesis and Resolution of Pain. Neuron. 2018;100(6):1292-1311.
15. Matisz CE, Gruber AJ. Neuroinflammatory remodeling of the anterior cingulate cortex as a key driver of mood disorders in gastrointestinal disease and disorders. Neurosci Biobehav Rev. 2022;133:104497.
16. Orock A, Johnson AC, Mohammadi E, Greenwood-Van Meerveld B. Environmental enrichment reverses stress-induced changes in the brain-gut axis to ameliorate chronic visceral and somatic hypersensitivity. Neurobiol Stress. 2024;28:100590.
17. Allen HN, Bobnar HJ, Kolber BJ. Left and right hemispheric lateralization of the amygdala in pain. Prog Neurobiol. 2021;196:101891.
18. Littmann ER, Lee JJ, Denny JE, Alam Z, Maslanka JR, Zarin I, et al. Host immunity modulates the efficacy of microbiota transplantation for treatment of Clostridioides difficile infection. Nat Commun. 2021;12(1):755.
19. Jensen SN, Cady NM, Shahi SK, Peterson SR, Gupta A, Gibson-Corley KN, et al. Isoflavone diet ameliorates experimental autoimmune encephalomyelitis through modulation of gut bacteria depleted in patients with multiple sclerosis. Sci Adv. 2021;7(28).
20. Ji G, Yakhnitsa V, Kiritoshi T, Presto P, Neugebauer V. Fear extinction learning ability predicts neuropathic pain behaviors and amygdala activity in male rats. Mol Pain. 2018;14:1744806918804441.
21. Zimmermann M. Ethical guidelines for investigations of experimental pain in conscious animals. Pain. 1983;16(2):109-110.
22. Ho Kim S, Mo Chung J. An experimental model for peripheral neuropathy produced by segmental spinal nerve ligation in the rat. Pain. 1992;50(3):355-363.
23. Bolyen E, Rideout JR, Dillon MR, Bokulich NA, Abnet CC, Al-Ghalith GA, et al. Reproducible, interactive, scalable and extensible microbiome data science using QIIME 2. Nat Biotechnol. 2019;37(8):852-857.
24. Quast C, Pruesse E, Yilmaz P, Gerken J, Schweer T, Yarza P, et al. The SILVA ribosomal RNA gene database project: improved data processing and web-based tools. Nucleic Acids Res. 2013;41(Database issue):D590-D596.
25. Yilmaz P, Parfrey LW, Yarza P, Gerken J, Pruesse E, Quast C, et al. The SILVA and «All-species Living Tree Project (LTP)» taxonomic frameworks. Nucleic Acids Res. 2014;42(Database issue):D643-D648.
26. Hu Y, Satten GA, Hu YJ. LOCOM: A logistic regression model for testing differential abundance in compositional microbiome data with false discovery rate control. Proc Natl Acad Sci U S A. 2022;119(30):e2122788119.
27. Bonomo RR, Cook TM, Gavini CK, White CR, Jones JR, Bovo E, Zima AV, Brown IA, Dugas LR, Zakharian E, Aubert G, Alonzo F 3rd, Calcutt NA, Mansuy-Aubert V. Fecal transplantation and butyrate improve neuropathic pain, modify immune cell profile, and gene expression in the PNS of obese mice. Proc Natl Acad Sci U S A. 2020;117(42):26482-26493.
28. Ustianowska K, Ustianowski L, Machaj F, Goracy A, Rosik J, Szostak B, et al. The Role of the Human Microbiome in the Pathogenesis of Pain. Int J Mol Sci. 2022;23(21).
29. Shi H, Chen M, Zheng C, Yinglin B, Zhu B. Fecal Microbiota Transplantation Alleviated Paclitaxel-Induced Peripheral Neuropathy by Interfering with Astrocytes and TLR4/p38MAPK Pathway in Rats. J Pain Res. 2023;16:2419-2432.
30. Wei J, Zhang C, Zhang Y, Zhang W, Doherty M, Yang T, et al. Association Between Gut Microbiota and Symptomatic Hand Osteoarthritis: Data From the Xiangya Osteoarthritis Study. Arthritis Rheumatol. 2021;73(9):1656-1662.
31. Ji RR, Chamessian A, Zhang YQ. Pain regulation by non-neuronal cells and inflammation. Science. 2016;354(6312):572-577.
32. Ji RR, Nackley A, Huh Y, Terrando N, Maixner W. Neuroinflammation and Central Sensitization in Chronic and Widespread Pain. Anesthesiology. 2018;129(2):343-366.
33. Salter MW, Stevens B. Microglia emerge as central players in brain disease. Nat Med. 2017;23(9):1018-1027.
34. Grace PM, Tawfik VL, Svensson CI, Burton MD, Loggia ML, Hutchinson MR. The Neuroimmunology of Chronic Pain: From Rodents to Humans. J Neurosci. 2021;41(5):855-865.
35. Carlessi AS, Borba LA, Zugno AI, Quevedo J, Réus GZ. Gut microbiota-brain axis in depression: The role of neuroinflammation. Eur J Neurosci. 2021;53(1):222-235.
36. Fung 2017 Fung TC, Olson CA, Hsiao EY. Interactions between the microbiota, immune and nervous systems in health and disease. Nat Neurosci. 2017;20(2):145-155.
37. Rudzki & Maes 2020 Rudzki L, Maes M. The Microbiota-Gut-Immune-Glia (MGIG) Axis in Major Depression. Mol Neurobiol. 2020;57(10):4269-4295.
38. Kukkar A, Singh N, Jaggi AS. Attenuation of neuropathic pain by sodium butyrate in an experimental model of chronic constriction injury in rats. J Formos Med Assoc. 2014;113(12):921-928.
39. Caetano-Silva ME, Rund L, Hutchinson NT, Woods JA, Steelman AJ, Johnson RW. Inhibition of inflammatory microglia by dietary fiber and short-chain fatty acids. Sci Rep. 2023;13(1):2819.
40. Yang T, Rodriguez V, Malphurs WL, Schmidt JT, Ahmari N, Sumners C, Martyniuk CJ, Zubcevic J. Butyrate regulates inflammatory cytokine expression without affecting oxidative respiration in primary astrocytes from spontaneously hypertensive rats. Physiol Rep. 2018;6(14):e13732.
41. Wei H, Yu C, Zhang C, Ren Y, Guo L, Wang T, Chen F, Li Y, Zhang X, Wang H, Liu J. Butyrate ameliorates chronic alcoholic central nervous damage by suppressing microglia-mediated neuroinflammation and modulating the microbiome-gut-brain axis. Biomed Pharmacother. 2023;160:114308.
42. Tang AT, Choi JP, Kotzin JJ, Yang Y, Hong CC, Hobson N, et al. Endothelial TLR4 and the microbiome drive cerebral cavernous malformations. Nature. 2017;545(7654):305-310.
43. Duan L, Zhang XD, Miao WY, Sun YJ, Xiong G, Wu Q, et al. PDGFRbeta Cells Rapidly Relay Inflammatory Signal from the Circulatory System to Neurons via Chemokine CCL2. Neuron. 2018;100(1):183-200 e8.
44. Matsuda M, Huh Y, Ji RR. Roles of inflammation, neurogenic inflammation, and neuroinflammation in pain. J Anesth. 2019;33(1):131-139.
45. Santos JM, Wang R, Bhakta V, Driver Z, Vadim Y, Kiritoshi T, et al. Turmeric Bioactive Compounds Alleviate Spinal Nerve Ligation-Induced Neuropathic Pain by Suppressing Glial Activation and Improving Mitochondrial Function in Spinal Cord and Amygdala. Nutrients. 2023;15(20).
46. Mazzitelli M, Ponomareva O, Presto P, John J, Neugebauer V. Impaired amygdala astrocytic signaling worsens neuropathic pain-associated neuronal functions and behaviors. Front Pharmacol. 2024;15:1368634.
47. Yuan T, Manohar K, Latorre R, Orock A, Greenwood-Van Meerveld B. Inhibition of Microglial Activation in the Amygdala Reverses Stress-Induced Abdominal Pain in the Male Rat. Cell Mol Gastroenterol Hepatol. 2020;10(3):527-543.
48. Chen Z, Wei H, Sagalajev B, Koivisto A, Pertovaara A. Amygdaloid administration of tetrapentylammonium attenuates development of pain and anxiety-like behavior following peripheral nerve injury. Pharmacol Rep. 2019;71(1):54-60.
49. Lin B, Wang Y, Zhang P, Yuan Y, Zhang Y, Chen G. Gut microbiota regulates neuropathic pain: potential mechanisms and therapeutic strategy. J Headache Pain. 2020;21(1):103.
50. Camilleri M. Leaky gut: mechanisms, measurement and clinical implications in humans. Gut. 2019;68(8):1516-1526.
51. Lamas M, González-Mariscal L, Gutiérrez R. Presence of claudins mRNA in the brain. Selective modulation of expression by kindling epilepsy. Brain Res Mol Brain Res. 2002;104(2):250-254.
52. Shen CL, Wang R, Yakhnitsa V, Santos JM, Watson C, Kiritoshi T, et al. Gingerol-Enriched Ginger Supplementation Mitigates Neuropathic Pain via Mitigating Intestinal Permeability and Neuroinflammation: Gut-Brain Connection. Front Pharmacol. 2022;13:912609.
53. Wang R, Santos JM, Dufour JM, Stephens ER, Miranda JM, Washburn RL, et al. Ginger Root Extract Improves GI Health in Diabetic Rats by Improving Intestinal Integrity and Mitochondrial Function. Nutrients. 2022;14(20).
54. Presto P, Ji G, Junell R, Griffin Z, Neugebauer V. Fear Extinction-Based Inter-Individual and Sex Differences in Pain-Related Vocalizations and Anxiety-like Behaviors but Not Nocifensive Reflexes. Brain Sci. 2021;11(10):1339.
55. Pieretti S, Di Giannuario A, Di Giovannandrea R, Marzoli F, Piccaro G, Minosi P, Aloisi AM. Gender differences in pain and its relief. Ann Ist Super Sanita. 2016;52(2):184-189.
56. Coyle DE, Sehlhorst CS, Mascari C. Female rats are more susceptible to the development of neuropathic pain using the partial sciatic nerve ligation (PSNL) model. Neurosci Lett. 1995;186(2-3):135-138. doi: 10.1016/0304-3940(95)11304-f. PMID: 7777182.
57. Ma J, Li J, Qian M, He N, Cao Y, Liu Y, et al. The comprehensive pathophysiological changes in a novel rat model of postinflammatory visceral hypersensitivity. Faseb J. 2019;33(12):13560-13571.
58. Freitag TL, Hartikainen A, Jouhten H, Sahl C, Meri S, Anttila VJ, et al. Minor Effect of Antibiotic Pre-treatment on the Engraftment of Donor Microbiota in Fecal Transplantation in Mice. Front Microbiol. 2019;10:2685.
The Author(s) 2024